Ten years ago today, the discovery of the type of particle known as the “Higgs Boson” was announced. [What is this particle and why was its discovery important? Here’s the most recent Higgs FAQ, slightly updated, and a literary article aimed at all audiences high-school and up, which has been widely read.]
But the particle was first produced by human beings in 1988 or 1989, as long as 34 years ago! Why did it take physicists until 2012 to discover that it exists? That’s a big question with big implications.
What Took So Long?
You’d think it would be easy. A Higgs particle is a ripple in the Higgs field. This field is present, and switched on (i.e. it has a non-zero value), throughout the universe, providing the known elementary particles with their mass (more precisely, with their rest mass.) Why aren’t its ripples everywhere?
- The problem is that Higgs particles are gone in a flash. They almost instantly decay (i.e. are transformed) into other particles. That’s why, even though they were abundantly created during the Big Bang, they all vanished long ago, and aren’t just lying around on the beach, waiting to be collected. We particle physicists have to make our own, from scratch; and when we create one, we can only infer we did so by identifying the debris that emerges from its demise.
Okay; but still, we’ve been making these ripples at the Tevatron, a proton-antiproton collider, and the Large Hadron Collider [LHC], a proton-proton collider, since the 1980s. What took scientists so long?
- At these colliders, the debris from a decaying Higgs particle often looks essentially identical to debris created in other processes, which are far more abundant. Even though a Higgs particle’s been created, there’s no way to tell from its remnants. [In physics-speak, the “Higgs signal” (the various signs that Higgs particles are being created) is drowned in “background” (other processes whose debris looks very similar.)] The vast majority of Higgs particles we make disappear without any obvious trace, like a straw-colored needle in a haystack.
That’s why, in order to make their discovery, the ATLAS and CMS experiments at the LHC focused on those rare Higgs particles whose decay debris is relatively distinctive — no more than 1 in every 400. (I’ve told that story here.) And seeing just one example is not enough, because there is still too much background. Hundreds of Higgs particles had to be detected, and their distinctive character isolated. Consequently hundreds of thousands of Higgs particles were needed for the discovery. That’s why it was out of reach of the Tevatron experiments, and why it took the LHC experiments two years to do it.
Where Do We Stand?
Here in 2022, ATLAS and CMS are closing in on 10 million Higgs particles each, which has allowed them to study the Higgs particle and the Higgs field in much more detail than was possible ten years ago. Since we’ve learned so much since 2012, you might well ask:
- How sure are the experts that this is really the Higgs particle predicted by the “Standard Model” of particle physics, and why? [By Standard Model, we mean the equations that describe all the known particles, with the assumption that the Higgs field and particle are of the simplest possible type.]
- How unsure are they, and why?
Here’s why one might say that scientists are pretty confident that this is the Standard Model’s Higgs particle, or a very close facsimile. In the Standard Model, every known elementary particle P that has a mass (specifically, a rest mass) engages with the Higgs field two ways:
- P gets its rest mass from the Higgs field; the stronger the interaction of P with the Higgs field, the larger its rest mass;
- P can interact with a Higgs particle; the strength of this interaction can potentially be measured at the LHC, because it affects how Higgs particles are produced and/or how they can decay.
Because the Higgs particle is nothing but a ripple in the Higgs field, the strength of particle P’s interaction with the Higgs field should be the same as the strength of its interaction with the Higgs particle! And therefore, the Standard Model predicts, for each type of particle, the interaction strength measured using method (2) should be the same as that inferred using method (1).
What do the LHC experiments say? [Note added: these plots were updated today by ATLAS and CMS! Added at the very end of this post.] Take a look at the figure below, from CMS. The top plot shows, for six types of particles (muons, taus, bottom quarks, W bosons, Z bosons and top quarks), the particle’s rest mass [method (1)] is on the horizontal axis, and the measurements of its interaction with Higgs particles [method (2)] on the vertical axis. The Standard Model’s prediction is the dashed line. That the data (the colored points) all lie nearly on the line shows that the Standard Model’s prediction works remarkably well!
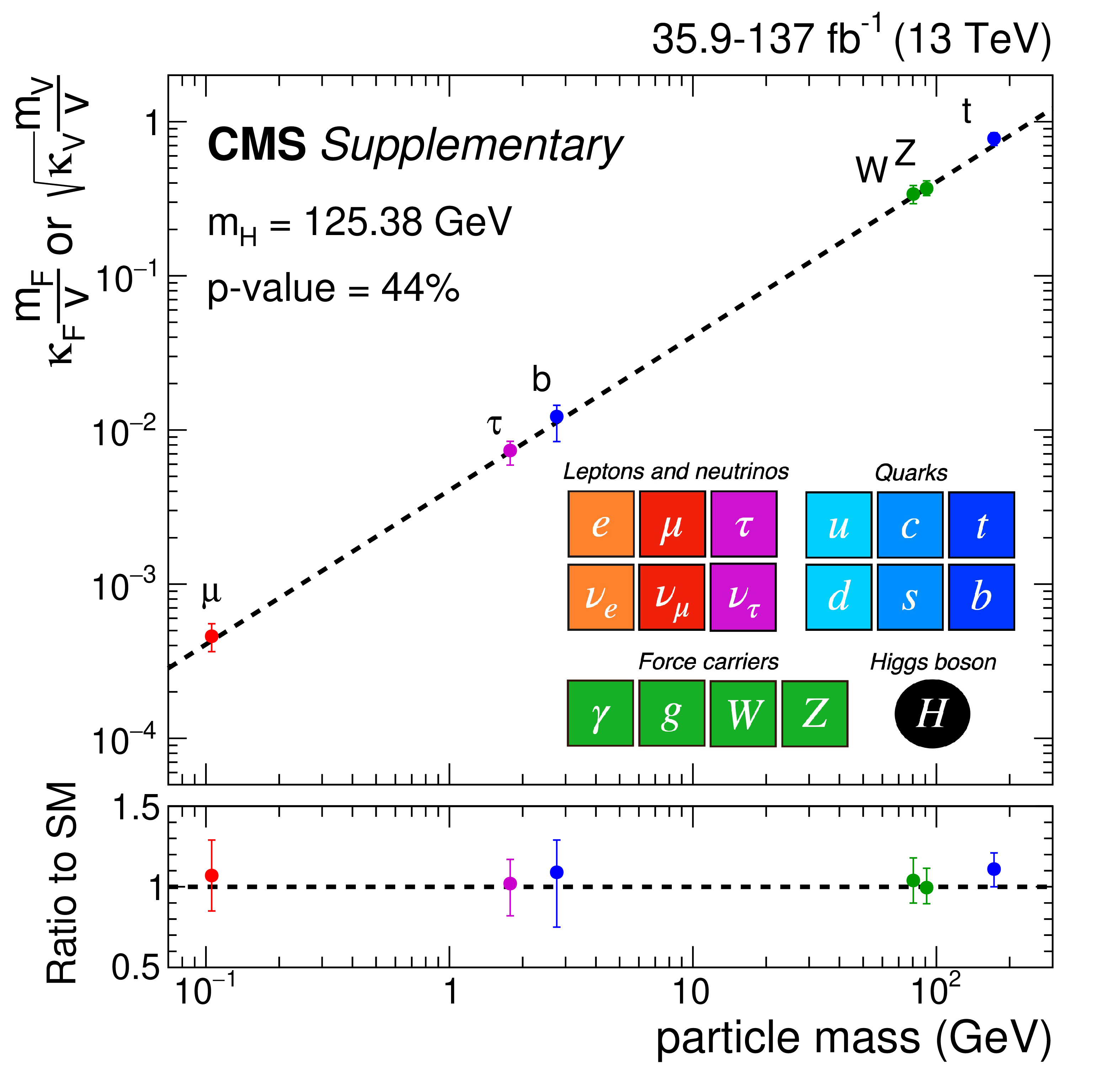
But how well, exactly? The bottom panel of the figure shows the excellent agreement of data with the Standard Model. If there were perfect agreement and perfect measurements, the points would lie at 1, but the measurements are still themselves imperfect, as indicated by the vertical bars, and so the deviations from 1 are within expectations. We can also see this same agreement on the left side of the next figure, from ATLAS.
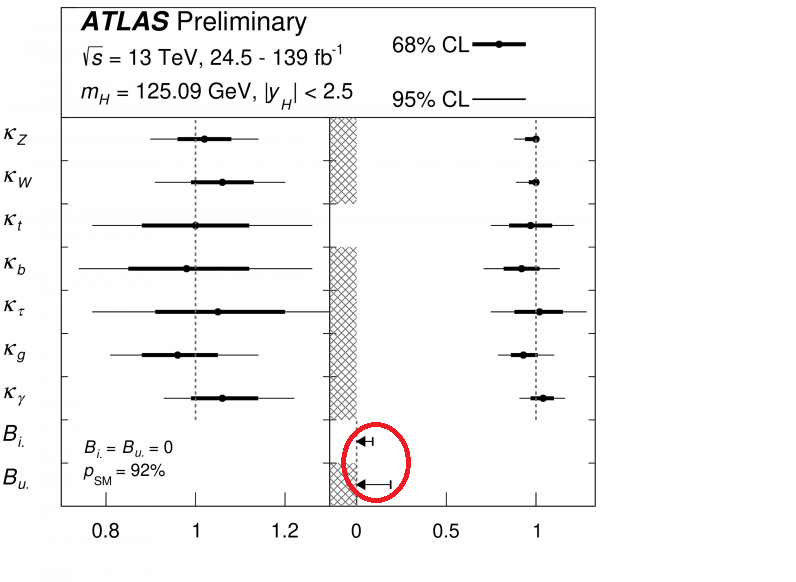
The right side of the figure then answers our second question: how unsure are scientists about whether this Higgs particle is that of the Standard Model? The answer, seen in the two circled bars at the bottom marked Bi and Bu, is in the 10-20% range. The specific questions they address are these:
- Could some Higgs particles decay to new particles which ATLAS and CMS cannot detect at all, as undetectable as neutrinos? Yes, according to the bar labeled Bi: perhaps as many as 1 in 10.
- Could some Higgs particles decay to new particles whose debris, while detectable by ATLAS and CMS, is lost in background and therefore currently hidden? Yes, according to the bar labeled Bu: perhaps as many as 1 in 5.
These are moderately small fractions, but enormous numbers. Remember that by now the LHC has made many millions of Higgs particles. For all we currently know, a million of these may have decayed to currently unknown particles — to particles not included in the Standard Model — without our having realized it yet. Even if it’s only a thousand which have done so, that possibility offers experimenters an opportunity that hasn’t been fully exploited.
This is why, if someone claims that “the LHC has confirmed the Standard Model and there’s nothing worth looking for in LHC data”, you might want to remind them that nature has the final word on that, and scientists ought to remain humble. The first Higgs particles were created in 1988, and had we not known how to look for them, they might have remained undiscovered well past 2012. Our colliders may have been creating non-Standard-Model particles for years or decades, and yet they may have remained undiscovered for technical reasons (they may still be too few or too difficult to observe at the LHC) or for sociological reasons (no one has yet done a careful search for them.)
How Best to Celebrate? With Two Hats
This is why I’m celebrating 34 years of Higgs boson production (and 10 years of knowing about it) in two very different ways.
Standard Model? Yes!
Wearing my 90%-Standard-Model hat, I’m preparing posts showing how the Standard Model’s main features can be understood with little or no technical knowledge. I’ll start each with an overview that requires no physics background, and then explain the details using just “dimensional analysis”, which I outlined in a few previous posts (here, here, here and here). No fancy math or quantum field theory needed!
Standard Model? No!
Wearing my 10%-Not-Standard-Model hat, meanwhile, I’m participating in a workshop of LHC theorists and experimenters that will focus on a little-studied phenomena within which non-Standard-Model particles could be hiding. The workshop topic is “Semi-Visible Jets”, a recent name for sprays of unknown, low-mass particles, of which some leave detectable debris inside ATLAS or CMS, while others escape undetected. [Disclaimer: I should reveal that I’m on the organizing committee. The local organizer and primary instigator is Annapaola de Cosa.]
Why wear both hats? Both have merits, and I feel it’s my job and responsibility to wear each one at least once a week. Even though the Standard Model is clearly the front-runner for explaining LHC data, and we need to think hard about the implications of its success, we also need to know, not assume, that LHC data agrees with it. At a collider like the LHC, new particles can escape our nets for a long time; the Higgs boson itself hid for almost 25 years. How can we be sure there isn’t something concealed in the LHC’s existing data, something that we could still discover? There’s only one way to find out: search as best we can.
Note Added: New Results Today from ATLAS and CMS!
New improved measurements today, using the full data set from Run 2, showing somewhat reduced uncertainties on some of the measurements, and first (very weak) limit on the interaction of the Higgs with the charm quark.
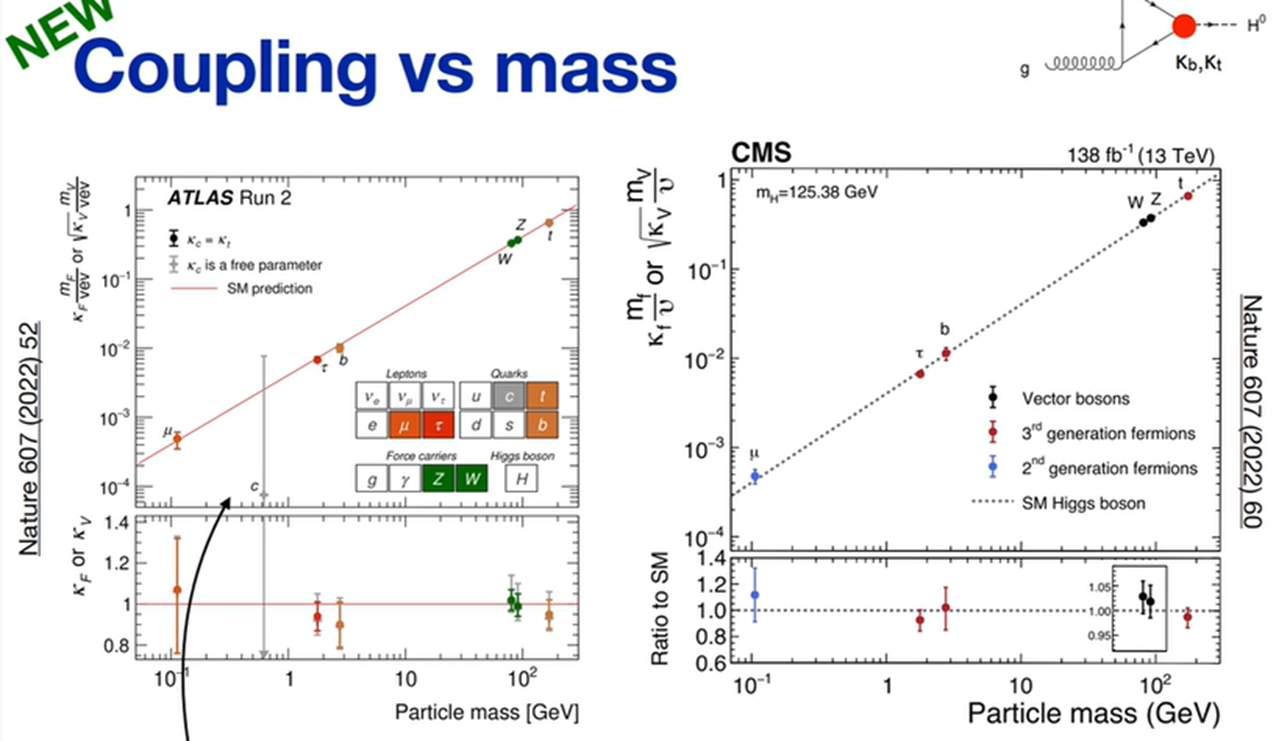
Also, some reduction in the amount of room for unnoticed new particles in Higgs decays, down from 20% toward 10-15%.
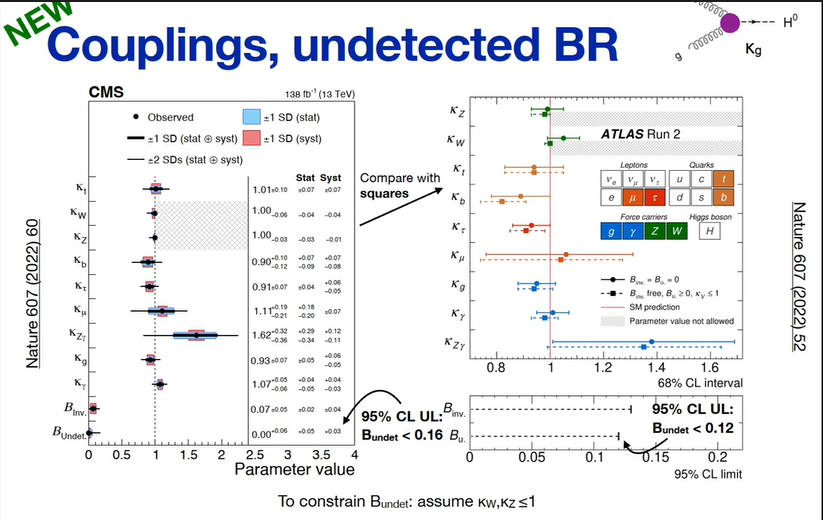
Tests of the Standard Model keep getting better! (Yet there’s still lots of room for hidden new physics, so there’s much more work to do.)
13 Responses
To stay alive you have to staple atom in the Human body an make a copy machine to live forever.
Thinking back a year earlier to 2011, I vividly remember Matthew commenting on the exhilarating pace at which various theoretical models and possible masses for the Higgs were already being ruled out by the summer results, leading to hints of the Higgs at 125Gev towards December. Exciting times, which must have been traumatizing for some theoretical physicists whose careers revolved around the development of their speculative models up to this point.
It was exciting, yes. But… not so traumatizing. Contrary to popular belief, especially the belief of crackpots who all have their own personal, highly developed theory of the universe, very few professional scientists,, especially the most prolific and creative ones, hinge their careers on a particular speculative model. To paraphrase Howard Georgi himself, who said as much at a conference I went to in my second year of graduate school, you make models not to be right but to gain understanding into what is possible. [Then he looked at Steve Weinberg and said, “Steve got lucky.” To which Weinberg responded, referring to the Standard Model, “I didn’t think it was right.” (His main reason, though he didn’t say so at the time: it didn’t work for quarks. Its prediction for strange mesons (Kaons) were wrong — until the charm quark was added in the early 1970s and fixed it all up.)]
What was more traumatizing was the discovery and confirmations in 2012-2013, which showed that the Higgs was very much Standard-Model-like. The banality of the new particle put the mindsets of entire communities of scientists on the chopping block, and forced an immense rethink that hasn’t fully played out yet.
34th Birthday? Not 34th Conception day?
The Higgs boson was conceived in a 1964 article by Peter Higgs.
No, I didn’t calculate at all. After all, the energy range is huge, and there are electrons, positrons, nuclei etc. Some energies are so high that there could be thousands or millions of cascade particles that could themselves generate a Higgs, etc. In the back of my mind of course was what a SUSY lover would think: “the energies are so high for some that cosmic rays just have to be able to make pairs of superparticles, if only we could detect them.”
Yeah, I think some of your statements aren’t correct. The energy range is huge, but the rate of cosmic rays is plunging versus energy, I think like E^2, and the center-of-mass energy E_cm is Sqrt[E] so you get E_cm^4, so you quickly stop benefitting by going to higher energy. Cosmic rays that can make Higgs bosons will be mostly protons; electrons, photons and neutrinos interact very weakly with Higgs bosons and you don’t produce that many scattering off atomic nuclei. So I think you’re conceptually overestimating how many will be made.
Real Higgses have been around, on Earth, for short times, since the Earth was created. They were created by collisions of energetic cosmic ray protons or other particles. Its just too infrequent to see them.
Have you actually done the calculation of the rate? It’s a little tricky and I can’t remember if I ever worked it out. Today’s back-of-envelope estimate is 1 per 100 years, but that could be off by a lot, given how carelessly I did it.
Who else than particle physicists are really interested in the Higgs boson?
Why do you ask? Apparently you’re interested enough to leave a comment. Clearly a lot of people are interested, or the world’s major newspapers wouldn’t have put the discovery on the front page. As for the fundamental importance of the Higgs field, and the importance of the discovery of the Higgs boson, see here https://profmattstrassler.com/articles-and-posts/the-higgs-particle/why-the-higgs-particle-matters/ . Whether you yourself choose to be interested in the universe, at least in those aspects on which your life depends, is a personal matter and is up to you.
I’m really confused. If, “the Higgs particle is nothing but a ripple in the Higgs field”, why call a “ripple” a “particle”?
Because in quantum physics, there is a “smallest possible ripple”, meaning a ripple of minimal amplitude (or height). That minimal ripple is called a “quantum” or a “particle”, but calling it a “particle” is indeed somewhat misleading, and the word “wavicle” is sometimes used instead. See https://profmattstrassler.com/articles-and-posts/the-higgs-particle/the-higgs-faq-2-0/ for some further discussion, and this series, https://profmattstrassler.com/articles-and-posts/particle-physics-basics/fields-and-their-particles-with-math/ , for some of the science, with a little bit of math (really not much) required.